- Food Security and Safety Focus Area, Faculty of Natural and Agricultural Science, North-West University, Mmabatho, South Africa
Genes possessed by microbes in the rhizosphere influence the metabolic activities that occur in this zone. Although the maize rhizosphere has been reported to be a hotspot of genes, these genes remain under-investigated. Hence, this study aimed at identifying putative microbial genes with plant beneficial functions in the underexplored maize rhizosphere microbiome using a shotgun metagenomics approach. Sampling was done at the flowering stage of the maize plants and both the rhizosphere and bulk soils were collected in triplicates. The metagenomes of the examined rhizosphere and bulk soils revealed genes involved in carbon fixation, nitrogen fixation, iron acquisition, heat and cold shock, phosphorus solubilization and utilization, sulfur cycling, and siderophore production. The beta diversity analysis showed significant variations (p < 0.05) in these genes across the examined rhizosphere and bulk soils which was further confirmed by the distinct separations between the samples as seen on the principal coordinate analysis (PCoA) plot. Contrarily, no significant difference was observed in diversity within the habitats (p = 0.99). The predominance of significant genes of agricultural importance such as the nifH, nifA, groES, and cspA in the rhizosphere metagenomes signifies that this region is endowed with beneficial organisms with potential for improving plant growth, mitigating stress, and reducing the effect of extreme temperatures, which can be optimized in developing biofertilizers. Therefore, the development of strategies that will help in cultivating these organisms, which are mostly unculturable, is encouraged. This would make them readily available for use as bio-inoculants and in other biotechnological applications.
Introduction
Maize (Zea mays) is one of the largest cultivated grain crops globally and an important staple crop with good nutritional value widely consumed in diverse processed forms (Huma et al., 2019). It is not only valuable for food consumption and indigenous purposes but highly applicable as raw material in industries for manufacturing various products (Rajoo 2021). Like any other crop, the community of microbes present in the maize rhizosphere makes up the food web network which uses the root exudates to regulate the diversity of microbes and activities going on in the region (Mendes et al., 2013).
The rhizosphere, which is the soil around the root in which several microbes reside (Omotayo and Babalola 2020; Zhou et al., 2020), is characterized by numerous anabolic and catabolic processes (Solís-García et al., 2021). The organisms in the rhizosphere perform various activities that induce nutrient cycling, promote a healthy plant, prevent biotic and abiotic stresses, and serve important functions in the developmental processes and well being of plants and crops like maize (Atkinson and Watson 2000; Sylvia and Chellemi 2001; Xu et al., 2009; Igiehon et al., 2019). Some of these microorganisms also act as defense for plants against phytopathogens, enhance growth through phytohormones production, and perform overall ecosystem functioning (Aremu et al., 2017).
It is imperative to acquire better understanding of the microbial genes that contribute to plant growth in the rhizosphere. The study of these genes will help us to know the ecological role which they play in the plant and the soil environment, realize their potential in sustainable agriculture, and also help to decipher their suitability in biotechnological applications (Li et al., 2014c). To date, studies are being carried out to identify and characterize microbial functional genes in agricultural soil environments, so as to unveil their input in agroecosystems (Li H. et al., 2014; Akinola et al., 2020b; Babalola et al., 2021). Nevertheless, with regards to the maize rhizosphere environment, especially in semi-arid regions, little or no studies have been conducted. This could be attributed to the limitations in the use of culture dependent techniques.
The emergence of high throughput sequencing methods, such as the shotgun metagenomic approach, which are culture independent have brought about more efficiency in analyzing various environments compared to the former culturing techniques. This is because of the accuracy of this technique in detecting a broad range of microbes and functional genes in a habitat (Enagbonma and Babalola 2019). In addition, the shotgun metagenomic approach is notable for eliminating the bias which may arise from using the traditional culturing methods (Lan et al., 2016).
Due to the need of characterizing functional genes of vital importance in the maize rhizosphere, efforts were made in this study to identify putative microbial genes which are beneficial to maize production in the underexplored maize rhizosphere and bulk soils using a shotgun metagenomic approach. Genomic analysis of these could further provide insight into the potential novelty of the genes. Moreover, awareness of the relationship between soil microbial distribution and function would contribute to and help in genetic engineering. We hypothesize that the rhizosphere soil will possess more abundant genes than the bulk soil and there will be variations in the gene composition of the rhizosphere soils due to differences in the microbial community structures and soil properties.
Materials and Methods
Sampling Site History
The selected sampling sites in Lichtenburg (25°59′40.8″S, 26°31′46.6″E) and Randfontein (26°11′51.3″S, 27°33′18.6″E) are maize growing areas in South-Africa which have been under intensive cultivation continuously for more than 10 years. They are both characterized with shrubs and grasses being a semi-arid region, the maize cultivar WE 3128 was planted on both sites. The average annual temperature and rainfall is around 16.9°C and 601 mm respectively in Lichtenburg while in Randfontein, it is around 15.3°C and 742 mm respectively. The type of soil found on the two farm sites can be classified as the Luvisol type based on the World reference base for soil resources classification (Anjos et al., 2015).
Sampling and Analysis of Soil Properties
After careful excavation of the maize plants, the roots were shaken by hand to remove soil loosely adhering to the roots, while the soil tightly attached to the roots was collected as rhizosphere soil. Bulk soils were collected from 0–15 cm depth, 10 m away from the cultivated area through a soil auger (Castañeda and Barbosa 2017; Enagbonma et al., 2019). Samples were collected in triplicates for both rhizosphere and bulk soils and were transported on ice (so as to prevent dehydration and degradation) to the laboratory immediately after collection for analysis. Soil samples were passed through a 2 mm sieve to remove unwanted particles and stored at −20°C until use for downstream applications.
The soil properties were measured using 20 g of air-dried soil from each sample, pH meter (EutechTM pH 150/Ph450 series) was used in measuring the soil pH in 1:2.5 soil water ratio (Springer 2014). A Gravimetric technique was used to determine the soil moisture as described by Shukla et al. (2014), and Morche’s (2008) method was used to determine sulfur concentration following extraction with 0.1 M hydrochloric acid (HCl). Total carbon and soil organic carbon were determined using the Santi et al. (2006) method, and the Walkey-Black procedures (Walkley and Black 1934) respectively.
The concentration of potassium in samples was measured following extraction with 1M ammonium acetate at pH of 7.0 (Jackson 1973). Bray and Kurtz’s protocol (Bray and Kurtz 1945) was used in measuring phosphorus concentration in samples, the described methods of Mussa et al. (2009) was used in determining soil nitrate, Potassium chloride (KCl) extraction method was used to analyze soil nitrate and ammonium (Reis et al., 2017), and the Loss-on-Ignition method was used to determine soil organic matter content following the procedure of Schulte and Hopkins (1996).
Molecular Analyses
Following manufacturer’s protocol, QIAGEN DNeasy PowerSoil Isolation Kit was used for the extraction of total DNA using 0.5 g of each soil sample. Following standard protocol, the concentration of DNA in samples were first examined using the Life Technologies Qubit® dsDNA HS Assay Kit. Nextera DNA Flex library preparation kit (Illumina) was used to prepare the libraries using about 50 ng DNA. Simultaneous fragmentation and addition of adapter sequences were done on the extracted DNA. The final concentrations of the prepared libraries were measured using the Qubit® dsDNA HS Assay Kit (Life Technologies), and the average library size was determined using the Agilent 2100 Bioanalyzer (Agilent Technologies). The libraries were pooled and diluted to 0.6 nM and sequenced paired end for 300 cycles using the HiSeq system (Illumina). Sequencing was done at the Molecular Research Laboratory (MR DNA) LP, Shallowater Texas, USA.
Metagenomics Sequence Annotation and Data Analyses
Unassembled DNA sequences were annotated with the Metagenomics Rapid Annotation (MG-RAST) pipeline version 3.3 following the prescribed lead of Meyer et al. (2008). Raw sequences were uploaded to the MG-RAST online server at https://www.mg-rast.org (Meyer et al., 2008). Following quality control, sequence annotation was done using BLAT algorithm against the M5NR database (Wilke et al., 2012) which provides a non-redundant incorporation of numerous databases such as KEGG (Kyoto Encyclopedia of Genes and Genomes), UniProtKB (UniProt Knowledgebase), and GenBank. Microbial taxonomic and gene profiling were performed using the M5NR and SEED level Subsystems respectively. BlastX with an e-value cutoff of E < 1 × 10−5, the minimum identity of 60% and a maximum alignment length of 15 base pairs was used to detect hits. Data normalization was applied on the MG-RAST pipeline so as to suppress the experimental noise effect. Sequences that failed annotation were left out in the analysis procedures. Carbon fixation, sulfur cycling, phosphorus utilization, nitrogen fixation, and other plant beneficial genes were categorized from the entire metagenomes obtained.
Statistical Analysis
The average values of the relative abundance of genes obtained from the three replicate samples from each sampling site were used for data analysis. Following normalization of the dataset, the rarefaction curve was plotted using the analysis tool in the MG-RAST server. The differences in the soil properties between the soil samples were analyzed using one-way analysis of variance with Tukey’s pairwise comparison test for significance level (p < 0.05) (Gunsalus et al., 2016). Assumptions (normality and homogeneity of variance) were determined and met. PAST (Paleontological Statistics) statistical software version 3.2 was used to evaluate the alpha diversity indices (Evenness, Simpson and Shannon indices) and Kruskal-Wallis test was used to determine the significance level (Hammer et al., 2001). Beta diversity was analyzed using one-way analysis of Similarities (ANOSIM) via 999 permutations and further illustrated by principal coordinate analysis (PCoA) based on Euclidean distance-matrix. (Lee et al., 2016). Data representation on the Principal coordinate analysis (PCoA) plot was done using the Canoco 5.12 software. Circos online software (www.circos.ca) was used in generating the chart for the gene abundance (Krzywinski et al., 2009). Representation and visualization of microbial taxonomy at the family level was carried out with a graph made using the Microsoft Excel software, version 2013. Quality sequences are deposited and available from NCBI SRA dataset under the accession number PRJNA678469 and PRJNA678475.
Results
Physical and Chemical Properties of Soil
As indicated in Table 1, the pH of R1 (Rhizosphere soil samples from Randfontein) and R2 (bulk soil samples from Randfontein) are 6.54 and 5.89 respectively, which differ significantly from that of F1 (rhizosphere samples from Lichtenburg) and F2 (bulk soil samples from Lichtenburg) which are 6.76 and 6.70 respectively. Sulphur and total carbon concentration did not differ significantly between R1 and R2, while a significant difference was seen in these two between F1 and F2. Potassium (K), N-NO3 and N-NH4 were significantly higher in the rhizosphere soils than their corresponding bulk soils. The concentration of organic carbon varied significantly between F1 and F2, while no significant difference was seen between R1 and R2. No significant difference was seen in the moisture content across the samples, however in the concentration of organic matter there was a significant difference between F1 and F2, while R1 and R2 did not differ significantly. There was no significant variation in the phosphorus content of F1 and F2, while in R1 and R2, these differ significantly.
The majority of the sequence read reached saturation, signifying that adequate data was obtained from the samples (Figure 1). The average number of sequences uploaded was 18, 942,494, 18,442,769, 15,666,716, and 15,045,869 for the rhizosphere soil samples from Lichtenburg (F1), bulk soil samples from Lichtenburg (F2), rhizosphere samples collected in Randfontein (R1), and bulk soil samples collected in Randfontein (R2) respectively. After quality control (QC), quality sequences that were retained in samples were 17,309,422, 16,779,302, 14,404,078, and 13,867,146 in F1, F2, R1, and R2 respectively with GC (Guanine-Cytosine) content of 65% in F1, F2, R1, and 66% in R2. Sequences with known protein functions were 5,391,241, 5,549,864, 4,763,335, and 4,954,381, for F1, F2, R1, and R2 respectively.
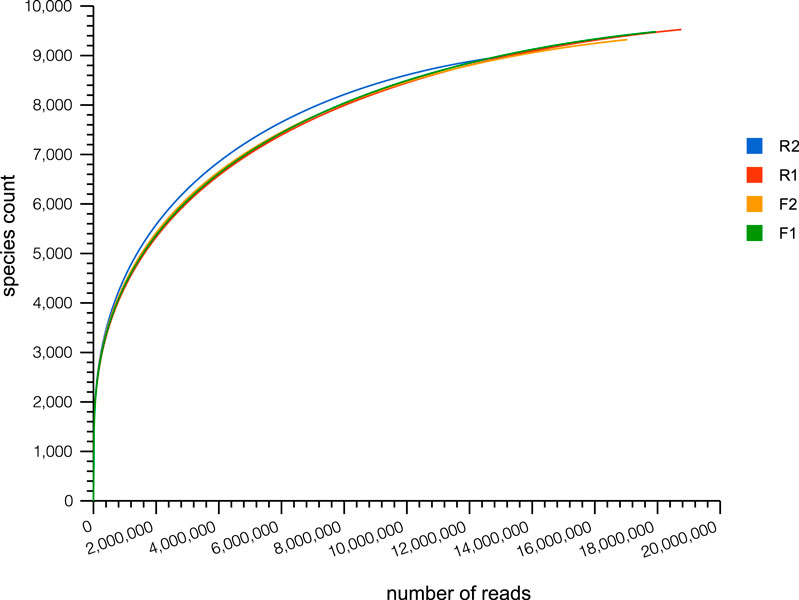
FIGURE 1. Rarefaction curve showing the average sequence reads in samples. F1 = 17,309,422; F2 = 16,779,302; R1 = 14,404,078; R2 = 13,867,146. F1 = maize rhizosphere samples from Lichtenburg, F2 = bulk soil samples from Lichtenburg, R1 = Rhizosphere soil samples from Randfontein, R2 = bulk soil samples from Randfontein.
Distribution of Microbial Communities in Samples
The dominant microbial class observed in the samples include Actinobacteria, Alphaproteobacteria, Betaproteobacteria, Deltaproteobacteria, Gammaproteobacteria, Planctomycetacia, Gemmatimonadetes, Solibacteres, Verrucomicrobiae, and Bacilli (Figure 2A). The dominant microbial families in the samples include Conexibacteraceae, Nitrospiraceae, Rubrobacteraceae, Nocardioidaceae, Planctomycetaceae, Nocardiaceae, Solibacteraceae, Rhizobiaceae, Burkholderiaceae, Pseudomonadaceae, Cytophagaceae, and Rhodobacteraceae (Figure 2B). No significant variation was seen in the relative abundance of the microbes in the samples (p > 0.05).
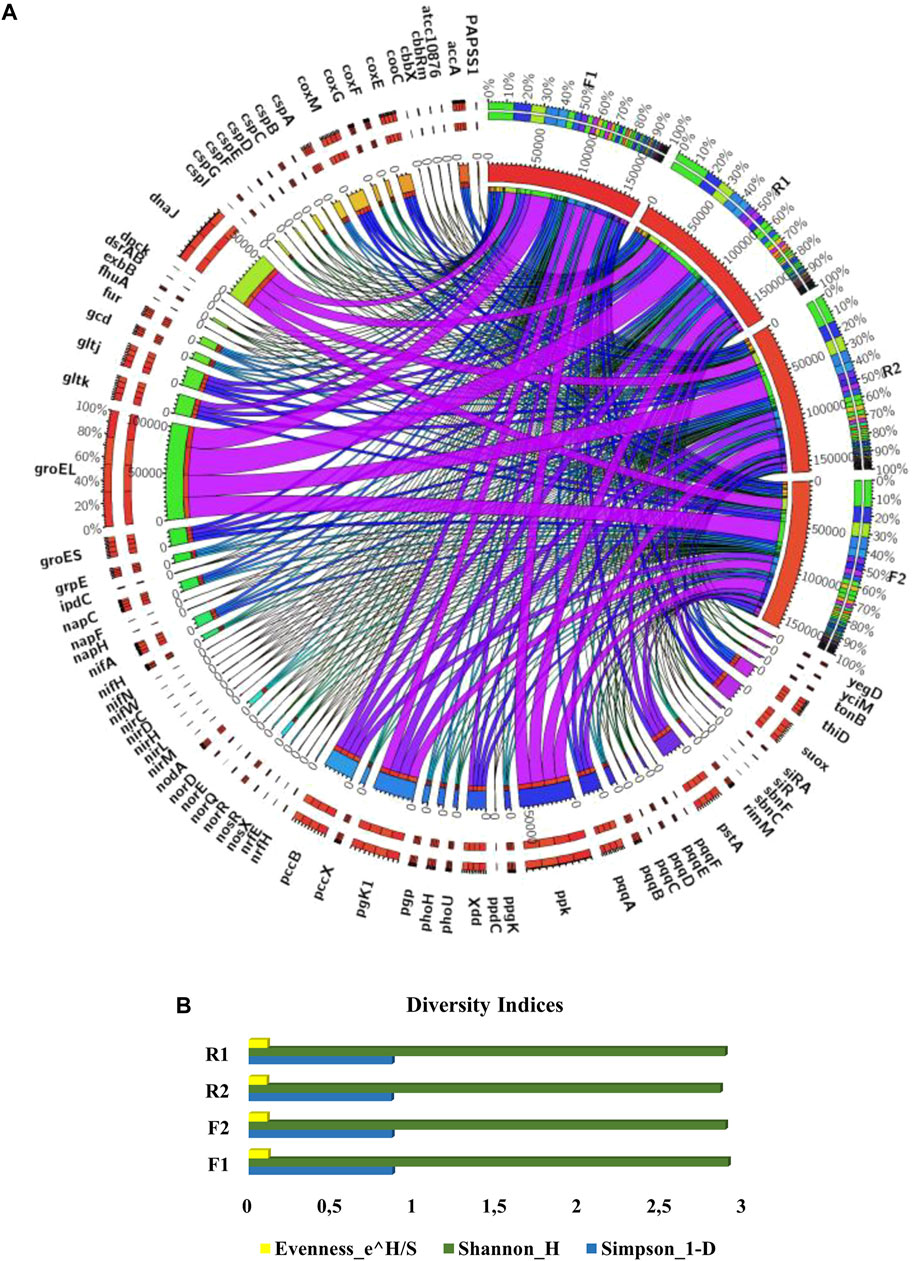
FIGURE 2. (A) Plant beneficial genes in the maize rhizosphere and bulk soil samples. F1 = maize rhizosphere samples from Lichtenburg, F2 = bulk soil samples from Lichtenburg, R1 = Rhizosphere soil samples from Randfontein, R2 = bulk soil samples from Randfontein. (B) Relative abundance of dominant microbial families observed in samples. F1 = maize rhizosphere samples from Lichtenburg, F2 = bulk soil samples from Lichtenburg, R1 = Rhizosphere soil samples from Randfontein, R2 = bulk soil samples from Randfontein.
Plant Growth Promoting/Nutrient Cycling Genes Identified in Samples
The metagenomic sequences obtained from the samples consist of genes involved in nutrient cycling (carbon fixation and degradation, nitrogen cycling, phosphorus utilization, and sulfur cycling) and other plant beneficial genes such as those involved in siderophore production, iron acquisition, phosphate solubilization, heat and cold shock (Figures 3, 4A,B). These genes aid plant growth and development and the presence of these in the maize rhizosphere indicated their contribution to maize growth.
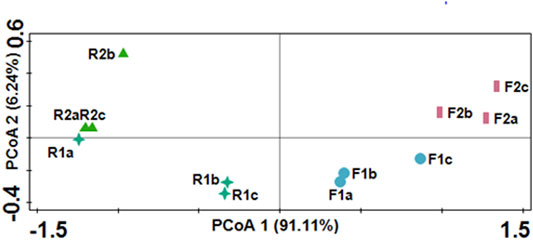
FIGURE 3. Plant beneficial genes in the maize rhizosphere and bulk soil samples. F1 = maize rhizosphere samples from Lichtenburg, F2 = bulk soil samples from Lichtenburg, R1 = Rhizosphere soil samples from Randfontein, R2 = bulk soil samples from Randfontein.
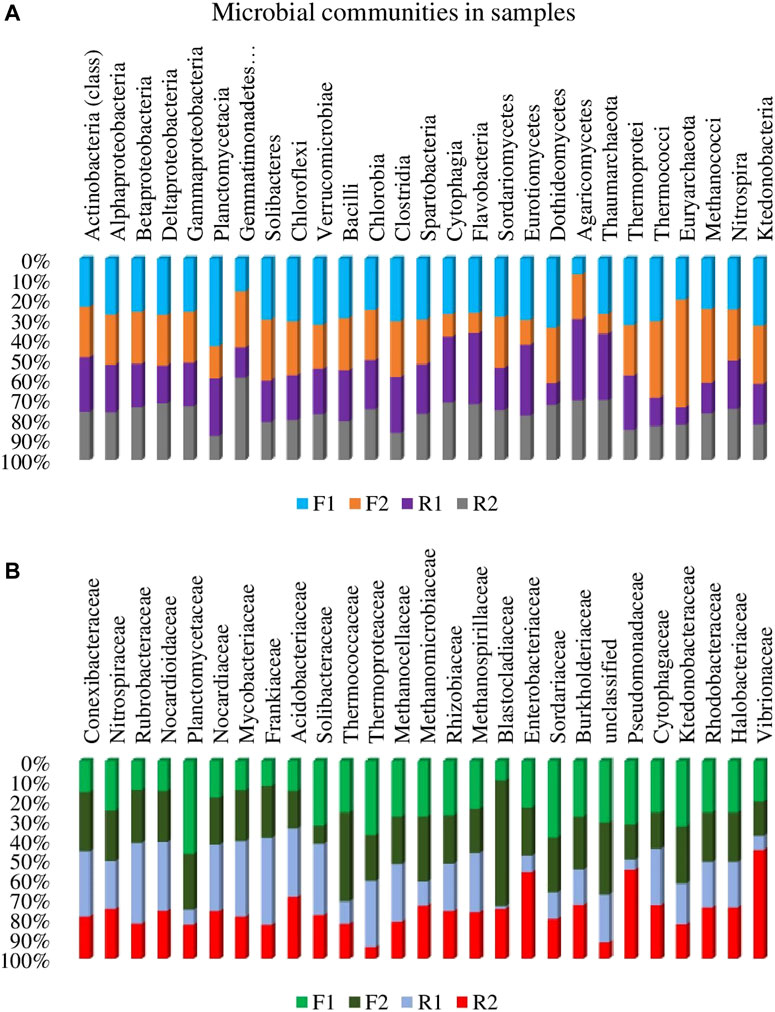
FIGURE 4. (A) Functional microbial genes in maize rhizosphere and its bulk soil. F1 = maize rhizosphere samples from Lichtenburg, F2 = bulk soil samples from Lichtenburg, R1 = Rhizosphere soil samples from Randfontein, R2 = bulk soil samples from Randfontein. (B) Plant beneficial genes in maize rhizosphere and its bulk soil, F1 = maize rhizosphere samples from Lichtenburg, F2 = bulk soil samples from Lichtenburg, R1 = Rhizosphere soil samples from Randfontein, R2 = bulk soil samples from Randfontein.
Diversity Estimation of Genes in the Samples
The alpha diversity analysis (diversity within the habitats) of the microbial genes showed no significant difference (p > 0.05) in the rhizosphere and bulk soil (Figure 5). However, the beta diversity analysis showed significant variations between the habitats (p < 0.05), this was further illustrated by the principal coordinate analysis which shows distinct separation between the samples on the PCoA plot (Figure 6).
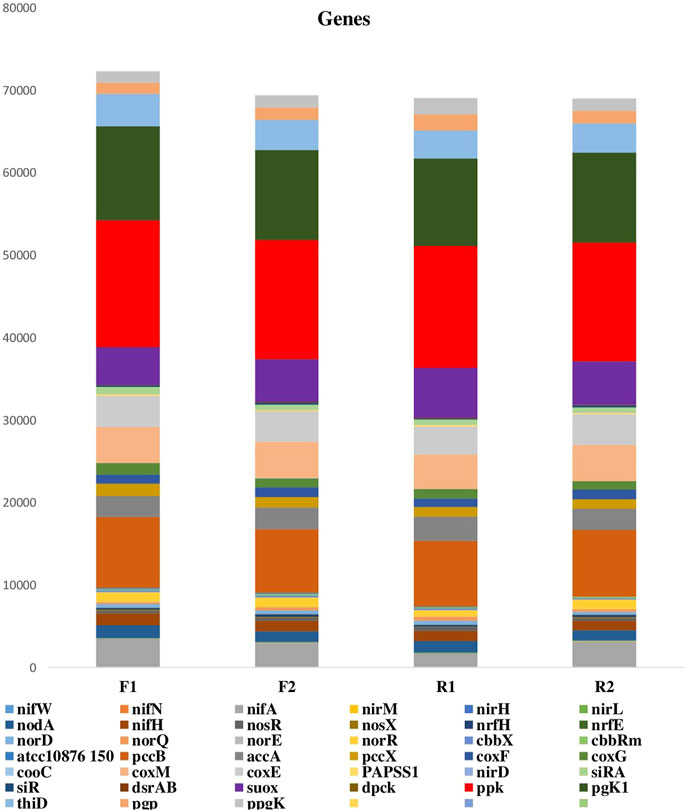
FIGURE 5. Alpha diversity indices of nutrient cycling genes in samples. F1 = maize rhizosphere samples from Lichtenburg, F2 = bulk soil samples from Lichtenburg, R1 = Rhizosphere soil samples from Randfontein, R2 = bulk soil samples from Randfontein.
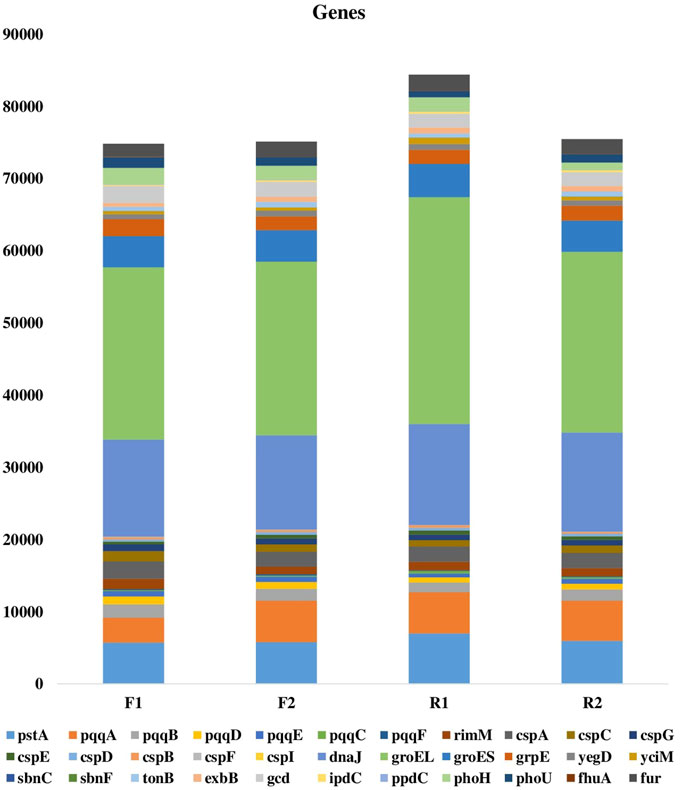
FIGURE 6. Principal coordinate analysis (PCoA) depicting the beta diversity in samples. F1 = maize rhizosphere samples from Lichtenburg, F2 = bulk soil samples from Lichtenburg, R1 = Rhizosphere soil samples from Randfontein, R2 = bulk soil samples from Randfontein.
Discussion
Some classes of microbes observed in the metagenomes such as Bacilli are notable for aiding plant growth (Ali 2021). They possess genes that are of immense benefits to plants (Li et al., 2014c) like those involved in the process of carbon fixation, nitrogen fixation, sulfur cycling, phosphate solubilization, iron acquisition, siderophore production and those responsible for reducing stress from environmental factors such as extreme temperatures and drought (Akinola et al., 2020b). Moreover, some of these microbes are able to enhance the secretion of 1-aminocyclopropane-1-carboxylate (ACC) which influences plant growth while some (Figures 2A,B) like Burkholderia cepacia which belongs to the Burkholderiaceae family contributes to plant growth indirectly by impeding plant disease and pathogens through production of siderophores and antibiotics (Kong et al., 2019).
Although diverse kinds of genes were unmasked in the metagenomes, our study focused on those involved in carbon fixation and degradation, nitrogen fixation, phosphorus utilization, sulfur cycling, phosphate solubilization, siderophore production, iron acquisition, and those responsible for heat and cold shock which are paramount and beneficial to the growth and development of maize plant in a semi-arid region.
Carbon Fixation and Degradation
Among the genes detected in this category are those which code for Ribulose-1,5-biphosphate carboxylase/oxygenase (RuBPco/RUBISCO, which is one of the enzymes involved in Calvin cycle), Propionyl-CoA carboxylase (Pcc, which catalyzes the carboxylation reaction of Propionyl CoA), and carbon monoxide dehydrogenase (CODH, involved in the reductive acetyl-CoA pathway in carbon cycle which allows organisms to make use of carbon monoxide as a source of energy and carbon (IV) oxide as a source of carbon (Li et al., 2014d)).
Genes associated with Ribulose-1,5-bisphosphate carboxylase/oxygenase (cbbX, cbbRm), carbon monoxide dehydrogenase (coxF, coxG, coxM, cooC, coxE) and Propionyl-CoA carboxylase (pccB, pccX) were seen to be more abundant in the rhizosphere soil samples (F1 and R1) than the bulk soils (Figures 3, 4A). In the rhizosphere samples, it however predominates F1 than R1 signifying a higher capacity for carbon fixation in this sample (Figures 3,4A) (Li et al., 2014c). Mechanisms of carbon fixation have been identified in the past in different species of Rhodobacteraceae (Rhodobacter sphaeroides, Rhodobacteraceae bacterium KLH11), Thermoproteaceae (Thermoproteus tenax), Thermococcaceae (Thermococcus onnurineus), Halobacteriaceae (Haloquadratum walsbyi), Pseudomonadaceae, and Vibrionaceae, (Dijkhuizen and Harder 1984; Berg et al., 2010; Li et al., 2014c; Pujalte et al., 2014), therefore the prevalence of these organisms in F1 (Figure 2B) justifies the abundance of these genes observed in the sample.
Phosphorus Utilization
As shown in Figures 3, 4A, Genes associated with inorganic polyphosphate degradation (ppx, ppgk), polyphosphate biosynthesis (pgk 1, ppk, thiD), dephospho-coenzyme A kinase (dpck), which catalyzes the ATP-dependent phosphorylation of dephospho-CoA in the biosynthesis of coenzyme A (CoA) were well represented in all the samples. The prevalence of these genes in F1 can be linked with the prevalence of Thermococcaceae, Rhizobiaceaea in this than R1 (Schut et al., 2014; Bechtaoui et al., 2019; Shimosaka et al., 2019). Also, the prevalence of ppx and ppgk in F1 suggests an intense polyphosphate transformation and more abundance of inorganic phosphorus (Li et al., 2014c) in the sample. Bacillariaceae was seen to predominate F1 rather than R1, some species of this bacteria family like the Bacillus cereus are known to be active in phosphorus metabolism. Bacillus cereus secretes different phospholipases which helps in phosphate retrieval metabolisms, it has been noted also for its ability to produce polyphosphate through the biodegradation of the herbicide glyphosate (Guddal et al., 1989; Acosta-Cortés et al., 2019).
Sulfur Cycling
The occurrence of siRA, suoX, papsS1, and dsrAB in the studied environments signifies the presence of microbes capable of metabolizing sulfur. siRA, genes were profound in F1 than other samples, thus indicating a high rate of sulfur transformation in F1 than R1 and other samples (Li et al., 2014c). Contrarily, genes involved with Sulfite oxidase and Sulfite reductase for oxidation and reduction of sulfur such as the suoX, papsS1 and dsrAB were more dominant in the R1 than F1 samples (Figure 4A).
Nitrogen Fixation
Genes involved in nitrogen fixation like the nitrite and nitrate reductase genes (nifN, nifA, nirD, napH, napC, napF) and those responsible for nitrite and glutamase transport (nirC, gltk and gltj), nitric and nitrous oxide (norQ and nosX), as well as the nirM, nirH, nirL were also revealed in the metagenomes (Figures 3, 4A). This can be affiliated with the occurrence of Rhodobacteraceae, Rhizobiaceae, Nitrospiraceae, Methanocellaceae, and Burkholderiaceae in the samples (Figure 2B). Species of these bacteria families such as Burkholderia vietnamiensis, Burkholderia cepacia, Agrobacterium fabrum (strain C58), Bradyrhizobium diazoefficiens (strain JCM 10833) Azotobacter vinelandii, and Klebsiella pneumoniae are associated with nitrogen fixation. The richness of F1 in nifH and nodA genes could be linked to the abundance of Rhizobiaceae, Burkholderiaceae in this sample as some species of this bacteria family like the Rhizobium leguminosarum, Paraburkholderia xenovorans, Cupriavidus basilensis, Cupriavidus necator are known for fixing nitrogen (Bournaud et al., 2017; da Silva et al., 2012; Estrada-de los Santos et al., 2011).
Genes Involved in Phosphate Solubilization, Heat Shock, Cold Shock, ACC Deaminase, and Siderophore Production
Furthermore, the metagenomes contain genes pqqABCDEF and gcd which play active roles in the biosynthesis of pyrroloquinoline quinone (PQQ) and transformation of insoluble phosphate to dissolved phosphate (soil phosphate solubilization), thus making it available for plant use (Otieno et al., 2015). According to Li L. et al. (2014) the above mentioned genes perform important roles in plant growth and biocontrol of crown gall disease caused by Agrobacterium tumefaciens.
Phosphate limitation in the soil triggers the autophosphorylation of histidine residue on sensory kinase, thus the presence of phoH and phoU complex which is involved in response to phosphate starvation and transport helps to control the metabolism and uptake of phosphate in response to phosphate limitation to active target sites (Akinola et al., 2020a). Occurrence of phoHU genes in all the metagenomes shows the ability of the resident soil microbes in enhancing limited soil phosphate. This is in line with the study of Myo et al. (2019) which showed that the effect of Streptomyces lividans TK24 in reshaping phosphate limited soil was as a result of the pho complex possessed by the organism. The occurrence of high affinity phosphate transporter pstA gene was also discovered in the metagenome.
Another hormone which is significant and essential for proper growth in plants is the Indoleacetic acid (IAA) (Myo et al., 2019). The ppdC and ipdC genes which code for phenylpyruvate decarboxylase and Indole-3-pyruvate decarboxylase which are the enzymes that produce Indole acetic acid from tryptophan (Jijón-Moreno et al., 2015) were found in the soil samples. Indole acetic acid assists in modulating numerous physiological processes like cell division, geotropisms, phototropism, root initiation, apical dominance and growth rate in plants (Singh and Dubey 2018; Carrión et al., 2019). The occurrence of the ipdC genes in the metagenomes can be associated with Enterobacter cloacae, a species of the Enterobacteriaceae family which occurred in the samples.
In the relationship between plant and bacteria, ACC deaminase reduces plant ethylenes notable for hindering the nodulation process (Kang et al., 2019). Genes that encode for ACC deaminase enzymes such as rimM were found more in the rhizosphere than bulk soil samples, this agrees with previous findings in which abundance of this gene was discovered in the rhizosphere than the bulk soil (Li et al., 2014c; Franco 2015).
Furthermore, stress alleviating genes such as the cspA, cspB, cspC, cspD, cspE, cspF, cspI, responsible for reducing cold-shock and groES, grpE, yegD, yciM, dnaJ which mitigates heat-shock were also discovered with more abundance in the rhizosphere soils. The higher abundance of heat shock genes in R1 shows that its microbiota demonstrates higher resistance to heat and stress caused as a result of drought. Drought is a major abiotic stress for plant especially in dry/arid regions like South Africa, it reduces plants potentials for growth and productivity (Nicolaes et al., 2014; Aydin et al., 2019). Moreover, the higher abundance of cold shock genes observed in the F1 than R1 sample shows the higher ability of its inherent microorganisms in cushioning plants against the harsh effect of downshift in temperature, thus aiding plant cells to adapt (Keto-Timonen et al., 2016). The occurrence of these stress reducing genes in our metagenomes shows the potentials of the sampling sites in reducing/alleviating the threat posed by heat and cold stress on plant/crop.
The fhuA gene which is a Ferrichrome-iron receptor in Escherichia coli and also a family of outer membrane proteins was seen in the samples. This gene together with the TonB-ExbB-ExbD which are the energy transducing complex of the cytoplasmic membrane are important in mediating the active transport of vitamin B12, siderophores and heme across the outer membrane of bacteria (especially gram-negative bacteria) (Zeng et al., 2013). In addition, the fhuA gene acts as a receptor for numerous bacteriophages, antibiotics and toxins which are harmful to bacterial cells. Rhizobium leguminosarum which belongs to the Alphaproteobacteria class are known to possess this gene (Carson et al., 2000; Akinola et al., 2020b), therefore the greater abundance of this class of bacteria in F1 than R1 and other samples, can be associated with the larger abundance of this gene in F1 than other samples.
The sbnF and sbnC genes are important in staphyloferrin B biosynthesis and are both associated with Staphylococcus aureus. The sbnF catalyzes the condensation of L-2,3-diaminopropionic acid (L-Dap) and citryl-diaminoethane to form L-2,3-diaminopropionyl-citryl-diaminoethane while the sbnC catalyzes the condensation of L-2,3-diaminopropionyl-citryl-diaminoethane and 2-oxoglutarate to form staphyloferrin B. The prevalence of these genes in F1 than R1 can be linked to the prevalence of Bacilli in this sample, since Staphylococcus aureus belongs to this bacteria class (Barka et al., 2016)
Environmental variables such as pH and the concentration of phosphorus, sulfur, total carbon and potassium, explains the distribution of plant beneficial microbes with plant growth promoting/beneficial genes in soil samples (Finn et al., 2020). As seen in this study, there were differences in the pH values of F1, F2, R1, and R2 (Table 1), sulfur concentrations varied significantly between F1 and F2, phosphorus differ significantly between R1 and R2, while between F1 and F2, significant difference was seen in potassium concentration and also between R1 and R2. The concentration of N-NO3 in the rhizosphere soils (8.52 and 17.09 in R1 and F1 respectively) showed that the biological processes which occur in these region are capable of improving growth in maize (Tale and Ingole 2015).
The substantial concentration of phosphorus and potassium in the rhizosphere samples indicated that immense physiological processes that are vital in the metabolism and biosynthesis of vital nutrients necessary for optimum growth in plants occur in their environments (Peralta et al., 2013; Akinola et al., 2020a).
The dissimilarities observed in the rhizosphere samples examined in this study could be linked to the peculiarities of the sampling location. Lichtenburg, where the F1 samples were collected is an agricultural area, while Randfontein, where R1 samples were collected comprises engineering industries and a gold mine. Thus, waste and residues such as heavy metals could have infiltrated into the soil, therefore, altering the physicochemical properties, community structure, and diversity of the soil microbes. Uranium extracted from gold ores is known for negatively impacting the soil microbial community structure and affecting genes such as those responsible for carbon and nitrogen cycling as seen in this study (Sutcliffe et al., 2017). Chromium is another metal associated with gold mines and has been known to alter the soil’s bacterial diversity (He et al., 2016). These heavy metals affect the soil microorganisms and interfere with soil microbial metabolisms.
The knowledge of soil microbial metabolisms is important in understanding interactions which enhance the ecosystem and improve plant growth and production (Jiménez et al., 2016; Igiehon and Babalola 2017; Enagbonma and Babalola 2020). This study revealed an ecologically diverse microbiome with nutrient cycling/plant growth-promoting traits. In the metagenomic reads, microbes such as Rhizobiaceae, Burkholderiaceae, Frankiaceae, and Pseudomonadaceae, Vibrionaceae were discovered. Certain strains of these bacteria families harbor genes which carry out nitrogen fixation, sulfur cycling, production of phytohormones (such as cytokinins, auxins, and gibberellins which help plants in nutrient uptake), inhibition of harmful pathogens and other growth and development processes in plants (Li et al., 2014d; Omotayo et al., 2019).
The alpha diversity indices showed that the microbial genes did not differ significantly within the habitats as revealed by Kruskal-Wallis test (p = 0.99). However, significant variation (p = 0.01) was seen in the beta diversity analysis which was determined using analysis of similarities (ANOSIM). This was further illustrated on the principal coordinate plot (Figure 6) on which there was a distinct separation between the soil samples. The strength of the separation (R) is 0.58, which depicts a clear difference between the samples. A notable distance was seen between the two rhizosphere soils (F1 and R1) which were also clearly distinguished from their corresponding bulk soils. The distance between each sample as revealed by the PCoA plot with a cumulative variation of 97.35% between the two axes (Figure 6) showed that the metagenomes are distinctly different in genetic structure.
The metadata obtained from this study showed and supports the notion that maize rhizosphere is a hotspot of genes accountable for converting labile and organic carbon, sulfur, nitrogen, and phosphorus compounds (Li et al., 2014c; Omotayo and Babalola 2020; Omotayo et al., 2021). This can be linked to the release of root exudates into the rhizosphere, as these rhizodeposits have a major influence on the rhizosphere microbiome, thus impacting its microbial diversity and functional profile, consequently affecting the microbial genes (Hu et al., 2018). Root exudates also possess the capacity to enhance latent soil organisms and activate their potentials. A wide range of compounds such as amino acids, lipids, and enzymes are present in the root exudates which influences the availability and wealth of potent genes in this region (Hu et al., 2018).
More potential to catabolize complex aromatic compounds was shown in the rhizosphere soils, especially R1 due to the abundance of Pseudomonadaceae in this sample (Jiménez et al., 2010; Li et al., 2014c). Strains of bacteria belonging to this family such as the P. putida KT2440, P. putida CSV86 are known for its ability to colonize the rhizosphere of various plants and degrade aromatic compounds (Basu et al., 2006; Jiménez et al., 2010).
Conclusion
The complex relationship that exists between the root and soil microorganisms propels the processes that occur within the rhizosphere. This study unveiled genes that express protein products needed for metabolizing carbon, nitrogen, phosphorus, iron, and sulfur. Other genes that perform crucial roles in phosphate solubilization, stress resistance, iron acquisition, heat, and cold shock were also discovered in the rhizosphere metagenomes. The distribution of the functional genes in the soil microbiomes considered in this study showed that variation exists between maize rhizospheres, which could be attributed to differences in the microbial structure which could have been influenced by the varying soil nutrient and majorly the root exudates. The abundance of genes vital for plant growth and production in the maize rhizosphere portrays this region to be endowed with microbes applicable as inoculants and in biofertilizer development, hence isolation of the microbes which possess these beneficial genes is hereby recommended due to their potential for improving soil quality, plant growth, and crop production.
Data Availability Statement
The datasets presented in this study can be found in online repositories. The names of the repository/repositories and accession number(s) can be found below: https://www.ncbi.nlm.nih.gov/, PRJNA678469; https://www.ncbi.nlm.nih.gov/, PRJNA678475.
Author Contributions
The study was conceived and conceptualized by OOB and OPO, while the bioinformatics and statistical analyses were done by OPO and ONI. OPO drafted the manuscript which was critiqued by ONI and OOB. All authors agreed on the final version of the manuscript before submission.
Conflict of Interest
The authors declare that the research was conducted in the absence of any commercial or financial relationships that could be construed as a potential conflict of interest.
Acknowledgments
OPO appreciate the North-West University, South Africa, for the enabled environment provided for the research. OOB acknowledged the National Research Foundation for grant (UID123634) which funds several research activities in her laboratory.
References
Acosta-Cortés, A. G., Martinez-Ledezma, C., López-Chuken, U. J., Kaushik, G., Nimesh, S., and Villarreal-Chiu, J. F. (2019). Polyphosphate Recovery by a Native Bacillus Cereus Strain as a Direct Effect of Glyphosate Uptake. Isme J. 13, 1497–1505. doi:10.1038/s41396-019-0366-3
Akinola, S. A., Ayangbenro, A. S., and Babalola, O. O. (2020a). The Diverse Functional Genes of Maize Rhizosphere Microbiota Assessed Using Shotgun Metagenomics. J. Sci. Food Agric. 101, 10948. doi:10.1002/jsfa.10948
Akinola, S. A., Ayangbenro, A. S., and Babalola, O. O. (2020b). The Diverse Functional Genes of Maize Rhizosphere Microbiota Assessed Using Shotgun Metagenomics. J. Sci. Food Agric. 6, 418–426. doi:10.1002/jsfa.10948
Ali, N. S. (2021). “Bacillus spp.: A Bio-Inoculant Factory for Plant Growth Promotion and Immune Enhancement,” in Biofertilizers (Amsterdam, Netherlands: Elsevier), 143–148. doi:10.1016/b978-0-12-821667-5.00021-x
Anjos, L., Gaistardo, C., Deckers, J., Dondeyne, S., Eberhardt, E., Gerasimova, M., et al. (2015). World Reference Base for Soil Resources 2014, Update 2015: International Soil Classification System for Naming Soils and Creating Legends for Soil Maps, Rome, Italy: FAO, 192.
Aremu, B. R., Alori, E. T., Kutu, R. F., and Babalola, O. O. (2017). “Potentials of Microbial Inoculants in Soil Productivity: An Outlook on African Legumes,” in Microorganisms for Green Revolution (Berlin, Germany: Springer), 53–75. doi:10.1007/978-981-10-6241-4_3,
Atkinson, D., and Watson, C. (2000). The Beneficial Rhizosphere: A Dynamic Entity. Amsterdam, Netherlands: Elsevier.
Aydin, M., Tombuloglu, G., Sakcali, M. S., Hakeem, K. R., and Tombuloglu, H. (2019). Boron Alleviates Drought Stress by Enhancing Gene Expression and Antioxidant Enzyme Activity. J. Soil Sci. Plant Nutr. 19, 545–555. doi:10.1007/s42729-019-00053-8
Babalola, O. O., Omotayo, O. P., and Igiehon, N. O. (2021). Survey of Maize Rhizosphere Microbiome Using Shotgun Metagenomics. Microbiol. Resour. Announc. 10. doi:10.1128/mra.01309-20
Barka, E. A., Vatsa, P., Sanchez, L., Gaveau-Vaillant, N., Jacquard, C., Klenk, H.-P., et al. (2016). Taxonomy, Physiology, and Natural Products of Actinobacteria. Microbiol. Mol. Biol. Rev. 80, 1–43. doi:10.1128/mmbr.00019-15
Basu, A., Apte, S. K., and Phale, P. S. (2006). Preferential Utilization of Aromatic Compounds over Glucose by Pseudomonas Putida CSV86. Appl. Environ. Microbiol. 72, 2226–2230. doi:10.1128/aem.72.3.2226-2230.2006
Bechtaoui, N., Raklami, A., Tahiri, A. I., Benidire, L., El Alaoui, A., Meddich, A., et al. (2019). Characterization of Plant Growth Promoting Rhizobacteria and Their Benefits on Growth and Phosphate Nutrition of Faba Bean and Wheat. Biol. Open 8. doi:10.1242/bio.043968
Berg, I. A., Kockelkorn, D., Ramos-Vera, W. H., Say, R. F., Zarzycki, J., Hügler, M., et al. (2010). Autotrophic Carbon Fixation in Archaea. Nat. Rev. Microbiol. 8, 447–460. doi:10.1038/nrmicro2365
Bournaud, C., Moulin, L., Cnockaert, M., Faria, S. d., Prin, Y., Severac, D., et al. (2017). Paraburkholderia Piptadeniae Sp. Nov. And Paraburkholderia Ribeironis Sp. nov., Two Root-Nodulating Symbiotic Species of Piptadenia Gonoacantha in Brazil. Int. J. Syst. Evol. Microbiol. 67, 432–440. doi:10.1099/ijsem.0.001648
Bray, R. H., and Kurtz, L. T. (1945). Determination of Total, Organic, and Available Forms of Phosphorus in Soils. Soil Sci. 59, 39–46. doi:10.1097/00010694-194501000-00006
Carrión, V. J., Perez-Jaramillo, J., Cordovez, V., Tracanna, V., De Hollander, M., Ruiz-Buck, D., et al. (2019). Pathogen-induced Activation of Disease-Suppressive Functions in the Endophytic Root Microbiome. Science 366, 606–612. doi:10.1126/science.aaw9285
Carson, K. C., Meyer, J.-M., and Dilworth, M. J. (2000). Hydroxamate Siderophores of Root Nodule Bacteria. Soil Biol. Biochem. 32, 11–21. doi:10.1016/s0038-0717(99)00107-8
Castañeda, L. E., and Barbosa, O. (2017). Metagenomic Analysis Exploring Taxonomic and Functional Diversity of Soil Microbial Communities in Chilean Vineyards and Surrounding Native Forests. PeerJ 5, e3098. doi:10.7717/peerj.3098
da Silva, K., Florentino, L. A., da Silva, K. B., De Brandt, E., Vandamme, P., and de Souza Moreira, F. M. (2012). Cupriavidus Necator Isolates Are Able to Fix Nitrogen in Symbiosis with Different Legume Species. Syst. Appl. Microbiol. 35, 175–182. doi:10.1016/j.syapm.2011.10.005
Dijkhuizen, L., and Harder, W. (1984). Current Views on the Regulation of Autotrophic Carbon Dioxide Fixation via the Calvin Cycle in Bacteria. Antonie Leeuwenhoek 50, 473–487. doi:10.1007/bf02386221
Enagbonma, B., and Babalola, O. (2019). Environmental Sustainability: A Review of Termite Mound Soil Material and its Bacteria. Sustainability 11, 3847. doi:10.3390/su11143847
Enagbonma, B. J., and Babalola, O. O. (2020). Unveiling Plant-Beneficial Function as Seen in Bacteria Genes from Termite Mound Soil. J. Soil Sci. Plant Nutr. 20, 1–10. doi:10.1007/s42729-019-00124-w
Enagbonma, B. J., Aremu, B. R., and Babalola, O. O. (2019). Profiling the Functional Diversity of Termite Mound Soil Bacteria as Revealed by Shotgun Sequencing. Genes 10, 637. doi:10.3390/genes10090637
Estrada-de los Santos, P., Vacaseydel-Aceves, N. B., Martínez-Aguilar, L., Cruz-Hernández, M. A., Mendoza-Herrera, A., and Caballero-Mellado, J. (2011). Cupriavidus and Burkholderia Species Associated with Agricultural Plants that Grow in Alkaline Soils. J. Microbiol. 49, 867–876. doi:10.1007/s12275-011-1127-9
Finn, D., Yu, J., and Penton, C. R. (2020). Soil Quality Shapes the Composition of Microbial Community Stress Response and Core Cell Metabolism Functional Genes. Appl. Soil Ecol. 148, 103483. doi:10.1016/j.apsoil.2019.103483
Franco, C. S. (2015). Isolation of Rhizobacteria from Salt Tolerant Plant Species and Evaluation of Their Plant Growth-Promotion. Giessen, Germany: Justus-Liebig-University.
Guddal, P. H., Johansen, T., Schulstad, K., and Little, C. (1989). Apparent Phosphate Retrieval System in Bacillus Cereus. J. Bacteriol. 171, 5702–5706. doi:10.1128/jb.171.10.5702-5706.1989
Gunsalus, R. P., Cook, L. E., Crable, B., Rohlin, L., McDonald, E., Mouttaki, H., et al. (2016). Complete Genome Sequence of Methanospirillum Hungatei Type Strain JF1. Stand Genomic Sci 11, 2. doi:10.1186/s40793-015-0124-8
Hammer, Ø., Harper, D. A., and Ryan, P. D. (2001). PAST: Paleontological Statistics Software Package for Education and Data Analysis. Palaeontol. Electron. 4, 9.
He, Z., Hu, Y., Yin, Z., Hu, Y., and Zhong, H. (2016). Microbial Diversity of Chromium-Contaminated Soils and Characterization of Six Chromium-Removing Bacteria. Environ. Manag. 57, 1319–1328. doi:10.1007/s00267-016-0675-5
Hu, L., Robert, C. A. M., Cadot, S., Zhang, X., Ye, M., Li, B., et al. (2018). Root Exudate Metabolites Drive Plant-Soil Feedbacks on Growth and Defense by Shaping the Rhizosphere Microbiota. Nat. Commun. 9, 2738–2813. doi:10.1038/s41467-018-05122-7
Huma, B., Hussain, M., Ning, C., and Yuesuo, Y. (2019). Human Benefits from Maize. Scholar J. Appl. Sci. Res. 2, 04–07.
Igiehon, N. O., Babalola, O. O., and Aremu, B. R. (2019). Genomic Insights into Plant Growth Promoting Rhizobia Capable of Enhancing Soybean Germination under Drought Stress. BMC Microbiol. 19, 159. doi:10.1186/s12866-019-1536-1
Igiehon, N. O., and Babalola, O. O. (2017). Biofertilizers and Sustainable Agriculture: Exploring Arbuscular Mycorrhizal Fungi. Appl. Microbiol. Biotechnol. 101, 4871–4881. doi:10.1007/s00253-017-8344-z
Jijón-Moreno, S., Marcos-Jiménez, C., Pedraza, R. O., Ramírez-Mata, A., de Salamone, I. G., Fernández-Scavino, A., et al. (2015). The ipdC, hisC1 and hisC2 Genes Involved in Indole-3-Acetic Production Used as Alternative Phylogenetic Markers in Azospirillum Brasilense. Antonie Leeuwenhoek 107, 1501–1517. doi:10.1007/s10482-015-0444-0
Jiménez, J., Nogales, J., García, J., and Díaz, E. (2010). “A Genomic View of the Catabolism of Aromatic Compounds in Pseudomonas,” in Handbook of Hydrocarbon and Lipid Microbiology (Berlin, Germany: Springer). doi:10.1007/978-3-540-77587-4_91
Jiménez-Bueno, N. G., Valenzuela-Encinas, C., Marsch, R., Ortiz-Gutiérrez, D., Verhulst, N., Govaerts, B., et al. (2016). Bacterial Indicator Taxa in Soils under Different Long-Term Agricultural Management. J. Appl. Microbiol. 120, 921–933. doi:10.1111/jam.13072
Kang, S. M., Shahzad, R., Bilal, S., Khan, A. L., Park, Y. G., Lee, K. E., et al. (2019). Indole-3-acetic-acid and ACC Deaminase Producing Leclercia Adecarboxylata MO1 Improves Solanum lycopersicum L. Growth and Salinity Stress Tolerance by Endogenous Secondary Metabolites Regulation. BMC Microbiol. 19, 80–14. doi:10.1186/s12866-019-1450-6
Keto-Timonen, R., Palonen, N., Hakakorpi, E., Lindström, A., Korkeala, M., and au, H. (2016). Cold Shock Proteins: a Minireview with Special Emphasis on Csp-Family of Enteropathogenic Yersinia. Front. Microbiol. 7, 1151. doi:10.3389/fmicb.2016.01151
Kong, H., Cheng, W., Wei, H., Yuan, Y., Yang, Z., and Zhang, X. (2019). An Overview of Recent Progress in Siderophore-Antibiotic Conjugates. Eur. J. Med. Chem. 182, 111615. doi:10.1016/j.ejmech.2019.111615
Krzywinski, M., Schein, J., Birol, İ., Connors, J., Gascoyne, R., Horsman, D., et al. (2009). Circos: an Information Aesthetic for Comparative Genomics. Genome Res. 19, 1639–1645. doi:10.1101/gr.092759.109
Lan, Y., Rosen, G., and Hershberg, R. (2016). Marker Genes that Are Less Conserved in Their Sequences Are Useful for Predicting Genome-wide Similarity Levels between Closely Related Prokaryotic Strains. Microbiome 4, 18–13. doi:10.1186/s40168-016-0162-5
Lee, S. A., Park, J., Chu, B., Kim, J. M., Joa, J.-H., Sang, M. K., et al. (2016). Comparative Analysis of Bacterial Diversity in the Rhizosphere of Tomato by Culture-dependent and -independent Approaches. J. Microbiol. 54, 823–831. doi:10.1007/s12275-016-6410-3
Li, H., Zhang, F., Rengel, Z., and Shen, J. (2014a). Rhizosphere Properties in Monocropping and Intercropping Systems between Faba Bean (Vicia faba L.) and Maize (Zea mays L.) Grown in a Calcareous Soil. Crop Pasture Sci. 64, 976–984.
Li, L., Jiao, Z., Hale, L., Wu, W., and Guo, Y. (2014b). Disruption of Gene pqqA or pqqB Reduces Plant Growth Promotion Activity and Biocontrol of Crown Gall Disease by Rahnella Aquatilis HX2. PLoS One 9, e115010. doi:10.1371/journal.pone.0115010
Li, X., Rui, J., Xiong, J., Li, J., He, Z., Zhou, J., et al. (2014d). Functional Potential of Soil Microbial Communities in the Maize Rhizosphere. PloS one 9, e112609–11269. doi:10.1371/journal.pone.0112609
Li, X., Rui, J., Xiong, J., Li, J., He, Z., Zhou, J., et al. (2014c). Functional Potential of Soil Microbial Communities in the Maize Rhizosphere. PloS one 9, e112609. doi:10.1371/journal.pone.0112609
Mendes, R., Garbeva, P., and Raaijmakers, J. M. (2013). The Rhizosphere Microbiome: Significance of Plant Beneficial, Plant Pathogenic, and Human Pathogenic Microorganisms. FEMS Microbiol. Rev. 37, 634–663. doi:10.1111/1574-6976.12028
Meyer, F., Paarmann, D., D'Souza, M., Olson, R., Glass, E. M., Kubal, M., et al. (2008). The Metagenomics RAST Server - a Public Resource for the Automatic Phylogenetic and Functional Analysis of Metagenomes. BMC Bioinforma. 9, 386–388. doi:10.1186/1471-2105-9-386
Morche, L. (2008). S-fluxes and Spatial Alterations of Inorganic and Organic Sulphur Fractions in Soil as Well as Their Accumulation and Depletion in the Rhizosphere of Agricultural Crops by Partial Use of the Radioisotope 35S. Bonn, Germany: Rheinischen Friedrich-Wilhelms-Universität Bonn.
Mussa, S. B., Elferjani, H. S., Haroun, F. A., and Abdelnabi, F. F. (2009). Determination of Available Nitrate, Phosphate and Sulfate in Soil Samples. Int. J. PharmTech Res. 1, 598–604.
Myo, E. M., Ge, B., Ma, J., Cui, H., Liu, B., Shi, L., et al. (2019). Indole-3-acetic Acid Production by Streptomyces Fradiae NKZ-259 and its Formulation to Enhance Plant Growth. BMC Microbiol. 19, 155–214. doi:10.1186/s12866-019-1528-1
Nicolaes, V., El Hajjaji, H., Davis, R. M., Van der Henst, C., Depuydt, M., Leverrier, P., et al. (2014). Insights into the Function of YciM, a Heat Shock Membrane Protein Required to Maintain Envelope Integrity in Escherichia coli. J. Bacteriol. 196, 300–309. doi:10.1128/jb.00921-13
Omotayo, O., Igiehon, O., and Babalola, O. (2021). Metagenomic Study of the Community Structure and Functional Potentials in Maize Rhizosphere Microbiome: Elucidation of Mechanisms behind the Improvement in Plants under Normal and Stress Conditions. Sustainability 13, 8079. doi:10.3390/su13148079
Omotayo, O. P., and Babalola, O. O. (2020). Resident Rhizosphere Microbiome's Ecological Dynamics and Conservation: Towards Achieving the Envisioned Sustainable Development Goals, a Review. Int. Soil Water Conservation Res. 9, 127–142. doi:10.1016/j.iswcr.2020.08.002
Omotayo, O. P., Omotayo, A. O., Mwanza, M., and Babalola, O. O. (2019). Prevalence of Mycotoxins and Their Consequences on Human Health. ToxicolRes 35, 1–7. doi:10.5487/tr.2019.35.1.001
Oteino, N., Lally, R. D., Kiwanuka, S., Lloyd, A., Ryan, D., Germaine, K. J., et al. (2015). Plant Growth Promotion Induced by Phosphate Solubilizing Endophytic Pseudomonas Isolates. Front. Microbiol. 6, 745. doi:10.3389/fmicb.2015.00745
Peralta, R. M., Ahn, C., and Gillevet, P. M. (2013). Characterization of Soil Bacterial Community Structure and Physicochemical Properties in Created and Natural Wetlands. Sci. Total Environ. 443, 725–732. doi:10.1016/j.scitotenv.2012.11.052
Pujalte, M. J., Lucena, T., Ruvira, M. A., Arahal, D. R., and Macián, M. C. (2014). The Family Rhodobacteraceae. Berlin, Germany: Springer.
Reis, C. R. G., Nardoto, G. B., Rochelle, A. L. C., Vieira, S. A., and Oliveira, R. S. (2017). Nitrogen Dynamics in Subtropical Fringe and Basin Mangrove Forests Inferred from Stable Isotopes. Oecologia 183, 841–848. doi:10.1007/s00442-016-3789-9
Santi, C., Certini, G., and D'Acqui, L. P. (2006). Direct Determination of Organic Carbon by Dry Combustion in Soils with Carbonates. Commun. soil Sci. plant analysis 37, 155–162. doi:10.1080/00103620500403531
Schulte, E., and Hopkins, B. (1996). Estimation of Soil Organic Matter by Weight Loss‐on‐ignition. Soil Org. matter Analysis interpretation 46, 21–31.
Schut, G. J., Lipscomb, G. L., Han, Y., Notey, J. S., Kelly, R. M., and Adams, M. M. W. (2014). “The Order Thermococcales and the Family Thermococcaceae,” in The Prokaryotes: Other Major Lineages of Bacteria and the Archaea (Berlin, Germany: Springer), 363–383. doi:10.1007/978-3-642-38954-2_324
Shimosaka, T., Makarova, K. S., Koonin, E. V., and Atomi, H. (2019). Identification of Dephospho-Coenzyme A (Dephospho-CoA) Kinase in Thermococcus Kodakarensis and Elucidation of the Entire CoA Biosynthesis Pathway in Archaea. Mbio 10, e01146–19. doi:10.1128/mBio.01146-19
Shukla, A., Panchal, H., Mishra, M., Patel, P., Srivastava, H., Patel, P., et al. (2014). Soil Moisture Estimation Using Gravimetric Technique and FDR Probe Technique: a Comparative Analysis. Am. Int. J. Res. Formal. Appl. Nat. Sci. 8, 89–92.
Singh, R., and Dubey, A. K. (2018). Diversity and Applications of Endophytic Actinobacteria of Plants in Special and Other Ecological Niches. Front. Microbiol. 9, 1767. doi:10.3389/fmicb.2018.01767
Solís-García, I. A., Ceballos-Luna, O., Cortazar-Murillo, E. M., Desgarennes, D., Garay-Serrano, E., Patiño-Conde, V., et al. (2021). Phytophthora Root Rot Modifies the Composition of the Avocado Rhizosphere Microbiome and Increases the Abundance of Opportunistic Fungal Pathogens. Front. Microbiol. 11, 3484. doi:10.3389/fmicb.2020.574110
Sutcliffe, B., Chariton, A. A., Harford, A. J., Hose, G. C., Greenfield, P., Elbourne, L. D. H., et al. (2017). Effects of Uranium Concentration on Microbial Community Structure and Functional Potential. Environ. Microbiol. 19, 3323–3341. doi:10.1111/1462-2920.13839
Sylvia, D. M., and Chellemi, D. O. (2001). Interactions Among Root-Inhabiting Fungi and Their Implications for Biological Control of Root Pathogens. Adv. Agron. 73, 1–33. doi:10.1016/S0065-2113(01)73003-9
Tale, K. S., and Ingole, S. (2015). A Review on Role of Physico-Chemical Properties in Soil Quality. Chem. Sci. Rev. Lett. 4, 57–66.
Walkley, A., and Black, I. A. (1934). An Examination of the Degtjareff Method for Determining Soil Organic Matter, and a Proposed Modification of the Chromic Acid Titration Method. Soil Sci. 37, 29–38. doi:10.1097/00010694-193401000-00003
Wilke, A., Harrison, T., Wilkening, J., Field, D., Glass, E. M., Kyrpides, N., et al. (2012). The M5nr: a Novel Non-redundant Database Containing Protein Sequences and Annotations from Multiple Sources and Associated Tools. BMC Bioinforma. 13, 141. doi:10.1186/1471-2105-13-141
Xu, Y., Wang, G., Jin, J., Liu, J., Zhang, Q., and Liu, X. (2009). Bacterial Communities in Soybean Rhizosphere in Response to Soil Type, Soybean Genotype, and Their Growth Stage. Soil Biol. Biochem. 41, 919–925. doi:10.1016/j.soilbio.2008.10.027
Zeng, X., Xu, F., and Lin, J. (2013). Specific TonB-ExbB-ExbD Energy Transduction Systems Required for Ferric Enterobactin Acquisition inCampylobacter. FEMS Microbiol. Lett. 347, 83–91. doi:10.1111/1574-6968.12221
Keywords: maize rhizosphere, microbes, microbial genes, sulfur cycling, iron acquisition, rhizosphere microbiome, heat and cold shock, shotgun metagenomics
Citation: Omotayo OP, Igiehon ON and Babalola OO (2022) Microbial Genes of Agricultural Importance in Maize Rhizosphere Unveiled Through Shotgun Metagenomics. Span. J. Soil Sci. 12:10427. doi: 10.3389/sjss.2022.10427
Received: 11 February 2022; Accepted: 24 May 2022;
Published: 05 July 2022.
Edited by:
David Fernández Calviño, University of Vigo, SpainCopyright © 2022 Omotayo, Igiehon and Babalola. This is an open-access article distributed under the terms of the Creative Commons Attribution License (CC BY). The use, distribution or reproduction in other forums is permitted, provided the original author(s) and the copyright owner(s) are credited and that the original publication in this journal is cited, in accordance with accepted academic practice. No use, distribution or reproduction is permitted which does not comply with these terms.
*Correspondence: Olubukola Oluranti Babalola, b2x1YnVrb2xhLmJhYmFsb2xhQG53dS5hYy56YQ==