Critical developmental periods of vulnerability in dystonia
- Department of Clinical and Experimental Epilepsy, UCL Queen Square Institute of Neurology, London, United Kingdom
Dystonia is a heterogenous movement disorder characterised by involuntary muscle contractions, leading to abnormal postures and movements. Despite being the third most common movement disorder, the pathophysiological mechanisms causing dystonia are incompletely understood. Isolated dystonia is often caused by pathogenic mutations in single genes. An emerging body of evidence suggests that at least some forms of isolated dystonia have a strong developmental component, with pathogenic effects acting within discrete periods of increased vulnerability during neurodevelopment. The extent to which this is a common feature of genetically distinct forms of dystonia, and which developmental mechanisms might be disrupted during these periods, remains unclear. During critical periods of development, neuronal activity is instructive in the maturation of neuronal circuits, and inappropriate levels of activity during this period can lead to permanent defects. This review, with an intentional focus on our work, outlines evidence implicating disruptions to neuronal activity during critical developmental periods as a potential mechanism underlying inherited motor disorders in general, and dystonia in particular.
Introduction
Dystonia is characterized by sustained or intermittent involuntary muscle contractions, which cause abnormal postures or movements [1]. Dystonia can occur through damage to the nervous system caused by trauma, stroke or neurodegenerative disease, or can be caused by pathogenic genetic mutations [2]. Studying how these mutations lead to dystonic movements is a powerful means to identify common molecular mechanisms involved in different forms of dystonia. An emerging body of evidence suggests that in some forms of genetic dystonia, particularly DYT1-TOR1A dystonia, the pathogenic effects of the dystonia-associated mutation may occur largely during critical periods of vulnerability during development [3] (discussed in Critical periods of vulnerability to genetic insult in dystonia section). This insight has far-reaching implications for our overall understanding of dystonia, and our approach towards therapeutic design. However, dystonia is a heterogenous disease, and a large number of genetically and phenotypically distinct forms have been described [2]. It is not clear to what extent this form of pathogenicity is shared across genetically distinct types of dystonia, or exactly what developmental processes are disrupted during critical periods of vulnerability.
A critical period of vulnerability to BK channel gain-of-function
Our work [4, 5] is focussed on a complex, early onset disorder characterised by dystonia and paroxysmal dyskinesia, caused by gain-of-function (GOF) mutations in the gene KCNMA1/SLO1, which encodes the α-subunit of the voltage- and Ca2+-sensitive BK (big K+) channel [6]. We investigate the novel hypothesis that GOF BK channels act during a critical developmental period of vulnerability to cause motor impairment, and interrogate the mechanisms by which they do so.
The first identified GOF variant was a dominant single base-pair mutation resulting in an aspartic acid to glycine change at residue 434 (D434G), which enhances the channel sensitivity to Ca2+ [7]. BK channels have a well-characterised role in modulating action potential waveform and neurotransmitter release [8, 9], and both LOF and GOF mutations are associated with neurodevelopmental phenotypes alongside motor dysfunction [10, 11]. Mouse models heterozygous for D434G exhibit alterations in both the firing rate and the dendritic and somatic morphology of cerebellar Purkinje neurons [12, 13]. Thus, it is equally plausible that BK channel GOF could cause dystonic and dyskinetic phenotypes either via acute changes to neurotransmission in circuits governing movement, or by disrupting neurodevelopment to produce lasting functional abnormalities.
We generated a Drosophila melanogaster (fruitfly) model heterozygous for a mutation equivalent to D434G in the highly conserved KCNMA1 orthologue, slowpoke (slo): sloE366G [4], hereafter referred to as “BKGOF.” This is an ideal system to investigate when during the lifecycle enhanced function of BK channels acts to produce motor impairment, as A) the powerful genetic toolkit available in Drosophila allows exquisite spatiotemporal control of gene expression, and B) unlike many rodent models of dystonia, the model exhibits robust motor defects. We thermogenetically restricted BKGOF expression to discrete periods of the fly life cycle, and tested for motor abnormalities using a machine-learning based video analysis software, the Feature Learning-based Limb segmentation and Tracking (FLLIT) system (Figures 1A, B) [14]. We narrowly defined a critical period during the final stages of neurodevelopment, coinciding with the final stages of synaptic maturation, during which only 24 h of BKGOF expression was sufficient to cause severe and permanent gait defects in the resulting adult flies [5]. In contrast, expression in the fully mature adult–for up to 5 days–had minimal or no effect on the movement parameters tested (Figure 1C) [5].
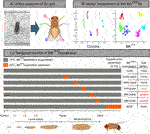
FIGURE 1. Identification of a critical period of vulnerability for BKGOF pathogenicity in Drosophila. (A) High speed videos of individual flies walking in a straight line are analysed using FLLIT, a machine-learning based software, which identifies and tracks the end point of each limb. (B) Representative FLLIT-derived traces showing the movement of each limb, relative to the body centre, over multiple strides. Control fly (left) movements are highly stereotyped and each limb has a clearly defined domain, while BKGOF flies’ movements are uncoordinated and irregular. (C) Schematic showing thermogenetic restriction of BKGOF expression during the fly life cycle. At 18°C expression is suppressed (blue) and at 29°C (red) permitted. Right: table summarizing the periods of BKGOF expression which cause the motor phenotype seen in (B).
Interestingly, we found that intrinsically-generated neuronal activity occurring during this critical period [15, 16] was suppressed, and that brain-wide expression of Bruchpilot—a key presynaptic active zone protein orthologous to mammalian ELKS [17]—was reduced during and after this critical period, but not before. We took two approaches to test whether this reduction in neuronal activity has a causal role in producing motor defects in BKGOF flies. First, we suppressed brain-wide neuronal activity in wild-type flies during development, using temporally restricted expression of a constitutively active open rectifying K+ channel, ORKΔC2, which hyperpolarises neurons and reduces their excitability [18]; intriguingly, this produced a motor phenotype strikingly similar to constitutive expression of BKGOF[5]. Secondly, we enhanced neuronal activity in BKGOF flies during the same period by transiently increasing activity of a population of relay neurons thought to drive neural activity during specific stages of development [16], using targeted expression of the temperature-sensitive cation channel TrpA1 [19]. This produced a partial rescue in the majority of the tested parameters [5]. We conclude that the pathogenic BK GOF mutation alters brain-wide neuronal activity levels during a critical developmental period, during which appropriate activity is instructive in establishing locomotor circuits, and this effect is at least partially responsible for the adult motor defects.
This mini-review will discuss critical developmental periods of vulnerability to dystonia-associated mutations, the instructive role of neuronal activity in the developing brain, and the plausibility of disruptions to this activity as a mechanism by which pathogenic mutations might act, with the aim of placing our work in the context of a wider field of research.
Critical periods of vulnerability to genetic insult in dystonia
The first indication that altered developmental processes contribute to dystonia came from observation of the patterns of disease inheritance and manifestation. DYT1-TOR1A dystonia, the first identified and most common form of inherited dystonia, is associated with mutations affecting the AAA-ATPase protein torsinA, the most common being a heterozygous 3 bp deletion (ΔGAG) with loss-of-function (LOF) and dominant-negative effects [20–22]. The mutations are partially penetrant, with only 30%–40% of carriers developing symptoms. These symptoms typically manifest in childhood, and if they have not developed by early adulthood typically will not appear later in life [20]. The most construct-valid mouse models of DYT1-TOR1A dystonia—heterozygous or homozygous for murine Tor1A-ΔGAG, or expressing the mutant form of human torsinA—have subtle motor defects but do not show overtly dystonia-like phenotypes [23–25]. However, a mouse model with conditional CNS-specific deletion of Tor1A exhibits a similar pattern to human TOR1A-ΔGAG carriers, with sensorimotor abnormalities and abnormal twisting postures manifesting during the first weeks of life, but not developing further as the mice age [26]. One explanation for this pattern is that a critical period of vulnerability exists early in life during which torsinA has a specific developmental role which can be disrupted by pathogenic mutations, but that if this does not occur, the same mutation is tolerated by the fully mature nervous system. Consistent with this hypothesis, juvenile Tor1A-ΔGAG/+ mice exhibit transient alterations in neuronal plasticity, morphology and glutamate receptor expression during a postnatal period analogous to the stage during which symptoms manifest in human patients [27], and in vitro evidence shows that nuclear envelope budding, a process associated with torsinA dysfunction, occurs during a discrete neurodevelopmental window and disappears as neurons fully mature [28]. A possible explanation for the critical period for disruption of torsinA function lies in the expression pattern of torsinA and the closely related protein torsinB. In mice null for both torsinA and torsinB, neuronal envelope buds develop earlier than in mice null for torsinA alone and persist into adulthood, and overexpression of either torsinA or torsinB is sufficient to rescue the budding phenotype caused by loss of torsinA in cultured cells [28]. Thus, the two proteins may have partially redundant functions, so that mutations disrupting torsinA function are compensated for during periods of high torsinB expression. Evidence from human tissue shows that torsinA and torsinB are detectable throughout the brain by around two postnatal months, and that expression of both is spatially and temporally restricted, consistent with specific roles in neurodevelopment [29, 30].
The question of when a given mutation acts during the life of an organism to produce a given phenotype can be most directly answered by placing the disease-causing mutation under spatiotemporal control (reviewed in Li et al [3]). Mouse models using this approach have demonstrated early-life critical periods of vulnerability to LOF of Ube3A [31, 32] (causing Angelman Syndrome) and Syngap1 [33] (causing SYNGAP1-related intellectual disability). Even the prototypical age-related disease Huntington’s Disease (HD) may fit into this pattern; restricting expression of a mutant form of huntingtin (htt) associated with HD to early life in a mouse model was sufficient to recapitulate a range of locomotor defects and neuropathological phenotypes associated with constitutive expression, many of which did not manifest until long after expression was suppressed [34]. Notably the pattern is not universal; phenotypes caused by LOF of Scn1a (associated with Dravet syndrome, a developmental encephelalopathy) and Mecp2 (associated with the neurodevelopmental disorder Rett Syndrome) are rescued by adult restoration of gene expression [35, 36], indicating that pathology stems from an ongoing effect of LOF in the mature nervous system. Recently, Li et al used this approach in a murine model to provide direct evidence for a critical period of vulnerability to torsinA LOF in early postnatal life [37]. Suppression of torsinA expression from embryogenesis onward recapitulated the behavioural and neuropathological phenotypes of constitutive LOF, while suppression in the fully mature animal alone did not [37]. The paper went on to demonstrate a critical therapeutic period; restoration of torsinA expression in juvenile mice was sufficient to rescue behavioural phenotypes, while restoration in the mature adult was not [37]. The authors conclude that torsinA LOF during a specific developmental period causes some pathogenic alteration in neurodevelopment that cannot be compensated for by normal function at a later stage.
These experiments elegantly demonstrate that the developing nervous system is uniquely vulnerable to certain pathogenic mutations during certain stages. While Li et al provide powerful evidence of a critical period of vulnerability to TorsinA LOF, the extent to which this feature is common to genetically distinct forms of dystonia is unknown. There is some evidence that other dystonia-causing mutations may also act during critical developmental periods [38]. For example, mutations in THAP1, associated with THAP1-dystonia, are also partially penetrant, and also cause early onset of symptoms [39]. Juvenile THAP1-dystonia model mice show temporary myelination defects which normalise in mature adults, while motor defects do not [40]. As in DYT1-TOR1A, this implies that some transient alteration in neurodevelopment causes permanent defects which cannot be compensated for in later life. Thus, acting during critical periods of vulnerability may be a common feature of dystonia-related mutations, but in most cases other than DYT1-TOR1A direct evidence is lacking. Further, the mechanisms underlying vulnerability during these stages are not understood.
Our work demonstrates that at least one other dystonia-associated mutation acts during a critical developmental period, and goes on to implicate alterations to neuronal activity during this period as a potential mechanism. The next section will discuss the role that neuronal activity plays during development, and the potential effects of disruptions to this activity.
Critical developmental periods
Maturation of neuronal circuits during development is often separated into two stages: early, activity-independent establishment of neural circuits (occurring before the onset of electrical neuronal firing and strongly determined by genetically programmed developmental processes); and later, activity-dependent refinement of these circuits (influenced by intrinsically-generated or stimulus-dependent neuronal activity) [41]. Critical developmental periods are understood to be discrete time periods during which neuronal activity has a far greater ability to influence the direction of neuronal maturation than at other stages, likely due to transient upregulation of activity-dependent plasticity mechanisms [42]. Appropriate input in the form of neuronal activity during these periods is necessary for the normal development of neuronal circuitry. Thus, alterations to neuronal development caused by inappropriate activity levels during these stages can become “locked in,” and cannot be fully compensated for by appropriate input later.
The paradigmatic example of a critical developmental period is the requirement of visual input in early life to establish the visual map. In a number of mammalian species, monocular deprivation (by reversibly suturing one eyelid closed) during a discrete postnatal period causes long lasting alterations in response to visual stimuli. Crucially, these changes persist when the eye is re-opened after the critical period, and similar changes are not produced by similar deprivation in the fully mature animal [43, 44]. This is not a phenomenon unique to the visual system. For example, monaural deprivation during critical periods has been shown to produce similar defects in the auditory system [45]. Critical periods in the development of the locomotor system are less well studied. However, reducing weight load on the limbs of neonatal rats during a well-defined postnatal period causes lasting alterations in locomotion, while the same interventions outside this window have less severe or transient effects [46, 47]. These experiments establish the key features of a critical period; 1) inappropriate neuronal activity during a discrete neurodevelopmental stage causes permanent behavioural defects, which 2) cannot be fully rescued by the resumption of appropriate neuronal activity after this stage, while 3) the same intervention is well tolerated by the fully mature nervous system and does not produce similar defects.
A large body of fundamental research has explored the mechanisms underlying the requirement of appropriate activity during discrete developmental stages. Neuronal activity levels during early development correlate with cell survival [48], synapse maturation [16, 49], and activity levels in mature neurons [50]. Importantly for the study of dystonia, activity during specific time periods seems to have a direct role in establishing locomotor circuits. Spontaneous activity occurs in the developing spinal cord of vertebrates [51], and disruptions cause motor neuron axonal pathfinding errors in developing chicks [52], delayed onset of co-ordinated motor-related activity in zebrafish [53], and altered left-right and flexor-extensor co-ordination in mice [54]. Evidence from Drosophila melanogaster larvae shows that perturbations to spontaneously generated activity during the embryonic stage causes lasting behavioural defects in the form of delayed and abnormal crawling behaviour [55, 56]. Experiments in both Drosophila and mice show that similar perturbations can also enhance seizure susceptibility, presumably reflecting long-lasting alterations to neuronal circuit function, and that normalising activity during the same stage can rescue these phenotypes [50, 57, 58].
Critical developmental periods therefore imply the existence of critical periods of vulnerability, as during these periods the system will be uniquely susceptible to alterations in patterns of neural activity across the brain.
Disrupted activity during developmental critical periods as a driver of human disease
As with animal models, humans have critical periods of enhanced susceptibility to genetic or environmental insults. For example, pre-natal exposure to alcohol can cause lasting developmental abnormalities in dosages that would be well tolerated in adulthood [59]. Other insults might be pathogenic at any stage of life. Understanding when and how a given insult affects the nervous system may have great therapeutic implications. For example, restoring gene function in adults may be a viable strategy in cases where a LOF mutation exerts a pathogenic function on the mature nervous system, but not when LOF during an earlier critical period has altered development in a way that will not be corrected by later normalisation of gene function.
Inappropriate levels of neuronal activity during critical developmental periods have been implicated in a number of conditions. Interventions for childhood disorders affecting eyesight must be performed at a young age to be fully effective [60], and cochlear implantation in congenitally deaf children has better long-term outcomes when performed at a younger age [61]. This is likely because of a requirement for appropriate sensory input during critical developmental periods, exactly as in animal studies of sensory deprivation. As well as sensory modalities, inappropriate levels of activity during critical periods has been theorised to underlie complex neurodevelopmental disorders such as autism and schizophrenia [62, 63].
Direct evidence that altered neuronal activity during a developmental critical period contributes to locomotor impairment in a human disease has recently come from a mouse model of HD in which neonatal mice display transient reductions in synaptic activity and changes in neuronal morphology, which normalise a few days later [64]. Pharmacologically enhancing glutamatergic neurotransmission during the same period to counteract this effect rectified motor phenotypes in resulting adult mice, providing strong evidence that the reductions in neuronal activity have a causal role in motor dysfunction. Interestingly, the same intervention worsened the performance of control mice in the same assays, underscoring the requirement for appropriate levels of activity during critical developmental periods [64]. Together with a wide body of fundamental research demonstrating the importance of appropriate neuronal activity during developmental critical periods in establishing locomotor circuits, this demonstrates that perturbations to this activity caused by pathogenic mutations is a potential causative mechanism underlying motor phenotypes in human disease. Not unlike the HD mice, juvenile Tor1A-ΔGAG/+ mice exhibit transient alterations in neuronal excitability and plasticity [27], though it is as yet unclear whether this has a causal role in behavioural phenotypes. Our work directly implicates alterations to neuronal activity during a critical developmental period as a mechanism underlying at least one form of inherited dystonia.
Discussion
An emerging body of work suggests that in at least some cases, pathogenic mutations causing dystonia act during critical neurodevelopmental periods of vulnerability. Our findings open the possibility that this may be a feature widely shared by genetically distinct forms of dystonia. Which developmental mechanisms are affected during these periods is an open question with far-reaching implications for understanding and treatment of dystonia. Disruption of any number of transient states or processes during neurodevelopment could cause long-term alterations to the maturation of the nervous system, and be a potential substrate for critical periods of vulnerability. One potential candidate is disruption of neuronal activity during critical periods of development. A wide body of research demonstrates that appropriate activity during critical periods is instructive in establishing locomotor circuits, and the mature system may not be able compensate for transient alterations to activity during these periods. Our work implicates altered neuronal activity during a critical developmental period as a causative mechanism underlying motor impairment in an invertebrate model of a pathogenic mutation directly associated with dystonia. In the context of the wider field, taking into account fundamental work, disease modelling, and clinical observation, we conclude that perturbations to neuronal activity levels during critical developmental periods is a potential mechanism that might underly various forms of inherited motor impairment, including dystonia.
Author contributions
SL was responsible for writing the first draft and revision of the final draft.
Conflict of interest
The author declares that the research was conducted in the absence of any commercial or financial relationships that could be construed as a potential conflict of interest.
Acknowledgments
I would like to acknowledge my mentors and colleagues, particularly Prof. James Jepson, Dr. Abigail Wilson, Dr. Gabriel Aughey, and Dr. Patrick Kratschmer, who contributed greatly to the research described here. I also thank the Dystonia Medial Research Foundation for funding the research described in the form of the 2020 Postdoctoral Fellowship Award, and for their ongoing support.
References
1. Albanese, A, Bhatia, K, Bressman, SB, Delong, MR, Fahn, S, Fung, VSC, et al. Phenomenology and classification of dystonia: a consensus update. Mov Disord (2013) 28:863–73. doi:10.1002/mds.25475
2. Weisheit, CE, Pappas, SS, and Dauer, WT. Inherited dystonias: clinical features and molecular pathways. Handbook Clin Neurol (2018) 147:241–54. doi:10.1016/B978-0-444-63233-3.00016-6
3. Li, J, Kim, S, Pappas, SS, and Dauer, WT. CNS critical periods: implications for dystonia and other neurodevelopmental disorders. JCI Insight (2021) 6:e142483. doi:10.1172/jci.insight.142483
4. Kratschmer, P, Lowe, SA, Buhl, E, Chen, KF, Kullmann, DM, Pittman, A, et al. Impaired pre-motor circuit activity and movement in a Drosophila model of KCNMA1-linked dyskinesia. Movement Disord (2021) 36:1158–69. doi:10.1002/mds.28479
5. Lowe, SA, Wilson, AD, Aughey, G, Banarjee, A, Goble, T, Simon-Batsford, N, et al. BK channel gain-of-function disrupts limb control by suppressing neurotransmission during a critical developmental window. Preprint (2023). Available from: https://doi.org/10.1101/2023.09.20.558625.
6. Du, W, Bautista, JF, Yang, H, Diez-Sampedro, A, You, SA, Wang, L, et al. Calcium-sensitive potassium channelopathy in human epilepsy and paroxysmal movement disorder. Nat Genet (2005) 37:733–8. doi:10.1038/ng1585
7. Díez-Sampedro, A, Silverman, WR, Bautista, JF, and Richerson, GB. Mechanism of increased open probability by a mutation of the BK channel. J Neurophysiol (2006) 96:1507–16. doi:10.1152/jn.00461.2006
8. Poolos, NP, and Johnston, D. Calcium-activated potassium conductances contribute to action potential repolarization at the soma but not the dendrites of hippocampal CA1 pyramidal neurons. J Neurosci (1999) 19:5205–12. doi:10.1523/JNEUROSCI.19-13-05205.1999
9. Griguoli, M, Sgritta, M, and Cherubini, E. Presynaptic BK channels control transmitter release: physiological relevance and potential therapeutic implications. J Physiol (2016) 594:3489–500. doi:10.1113/JP271841
10. Miller, JP, Moldenhauer, HJ, Keros, S, and Meredith, AL. An emerging spectrum of variants and clinical features in KCNMA1-linked channelopathy. Channels (Austin) (2021) 15:447–64. doi:10.1080/19336950.2021.1938852
11. Liang, L, Li, X, Moutton, S, Schrier Vergano, SA, Cogné, B, Saint-Martin, A, et al. De novo loss-of-function KCNMA1 variants are associated with a new multiple malformation syndrome and a broad spectrum of developmental and neurological phenotypes. Hum Mol Genet (2019) 28:2937–51. doi:10.1093/hmg/ddz117
12. Dong, P, Zhang, Y, Hunanyan, AS, Mikati, MA, Cui, J, and Yang, H. Neuronal mechanism of a BK channelopathy in absence epilepsy and dyskinesia. Proc Natl Acad Sci (2022) 119:e2200140119. doi:10.1073/pnas.2200140119
13. Mi, PS, Roache, CE, Iffland, PH, Moldenhauer, HJ, Matychak, KK, Plante, AE, et al. BK channel properties correlate with neurobehavioral severity in three KCNMA1-linked channelopathy mouse models. eLife (2022) 11:e77953. doi:10.7554/eLife.77953
14. Wu, S, Tan, KJ, Govindarajan, LN, Stewart, JC, Gu, L, Ho, JWH, et al. Fully automated leg tracking of Drosophila neurodegeneration models reveals distinct conserved movement signatures. Plos Biol (2019) 17:e3000346. doi:10.1371/journal.pbio.3000346
15. Akin, O, Bajar, BT, Keles, MF, Frye, MA, and Zipursky, SL. Cell-type-Specific patterned stimulus-independent neuronal activity in the Drosophila visual system during synapse formation. Neuron (2019) 101:894–904. doi:10.1016/j.neuron.2019.01.008
16. Bajar, BT, Phi, NT, Isaacman-Beck, J, Reichl, J, Randhawa, H, and Akin, O. A discrete neuronal population coordinates brain-wide developmental activity. Nature (2022) 602:639–46. doi:10.1038/s41586-022-04406-9
17. Wagh, DA, Rasse, TM, Asan, E, Hofbauer, A, Schwenkert, I, Dürrbeck, H, et al. Bruchpilot, a protein with homology to ELKS/CAST, is required for structural integrity and function of synaptic active zones in Drosophila. Neuron (2006) 49:833–44. doi:10.1016/j.neuron.2006.02.008
18. Nitabach, MN, Blau, J, and Holmes, TC. Electrical silencing of Drosophila pacemaker neurons stops the free-running circadian clock. Cell (2002) 109:485–95. doi:10.1016/s0092-8674(02)00737-7
19. Hamada, FN, Rosenzweig, M, Kang, K, Pulver, SR, Ghezzi, A, Jegla, TJ, et al. An internal thermal sensor controlling temperature preference in Drosophila. Nature (2008) 454:217–20. doi:10.1038/nature07001
20. Bressman, SB, Sabatti, C, Raymond, D, de Leon, D, Klein, C, Kramer, PL, et al. The DYT1 phenotype and guidelines for diagnostic testing. Neurology (2000) 54:1746–52. doi:10.1212/wnl.54.9.1746
21. Torres, GE, Sweeney, AL, Beaulieu, J-M, Shashidharan, P, and Caron, MG. Effect of torsinA on membrane proteins reveals a loss of function and a dominant-negative phenotype of the dystonia-associated ΔE-torsinA mutant. Proc Natl Acad Sci (2004) 101:15650–5. doi:10.1073/pnas.0308088101
22. Wakabayashi-Ito, N, Ajjuri, RR, Henderson, BW, Doherty, OM, Breakefield, XO, O'Donnell, JM, et al. Mutant human torsinA, responsible for early-onset dystonia, dominantly suppresses GTPCH expression, dopamine levels and locomotion in Drosophila melanogaster. Biol Open (2015) 4:585–95. doi:10.1242/bio.201411080
23. Sharma, N, Baxter, MG, Petravicz, J, Bragg, DC, Schienda, A, Standaert, DG, et al. Impaired motor learning in mice expressing TorsinA with the DYT1 dystonia mutation. J Neurosci (2005) 25:5351–5. doi:10.1523/JNEUROSCI.0855-05.2005
24. Dang, MT, Yokoi, F, McNaught, KS, Jengelley, TA, Jackson, T, Li, J, et al. Generation and characterization of Dyt1 ΔGAG knock-in mouse as a model for early-onset dystonia. Exp Neurol (2005) 196:452–63. doi:10.1016/j.expneurol.2005.08.025
25. Goodchild, RE, Kim, CE, and Dauer, WT. Loss of the dystonia-associated protein TorsinA selectively disrupts the neuronal nuclear envelope. Neuron (2005) 48:923–32. doi:10.1016/j.neuron.2005.11.010
26. Liang, C-C, Tanabe, LM, Jou, S, Chi, F, and Dauer, WT. TorsinA hypofunction causes abnormal twisting movements and sensorimotor circuit neurodegeneration. J Clin Invest (2014) 124:3080–92. doi:10.1172/JCI72830
27. Maltese, M, Stanic, J, Tassone, A, Sciamanna, G, Ponterio, G, Vanni, V, et al. Early structural and functional plasticity alterations in a susceptibility period of DYT1 dystonia mouse striatum. eLife (2018) 7:e33331. doi:10.7554/eLife.33331
28. Tanabe, LM, Liang, C-C, and Dauer, WT. Neuronal nuclear membrane budding occurs during a developmental window modulated by torsin paralogs. Cell Rep (2016) 16:3322–33. doi:10.1016/j.celrep.2016.08.044
29. Siegert, S, Bahn, E, Kramer, ML, Schulz-Schaeffer, WJ, Hewett, JW, Breakefield, XO, et al. TorsinA expression is detectable in human infants as young as 4 weeks old. Develop Brain Res (2005) 157:19–26. doi:10.1016/j.devbrainres.2005.02.019
30. Bahn, E, Siegert, S, Pfander, T, Kramer, ML, Schulz-Schaeffer, WJ, Hewett, JW, et al. TorsinB expression in the developing human brain. Brain Res (2006) 1116:112–9. doi:10.1016/j.brainres.2006.07.102
31. Silva-Santos, S, van Woerden, GM, Bruinsma, CF, Mientjes, E, Jolfaei, MA, Distel, B, et al. Ube3a reinstatement identifies distinct developmental windows in a murine Angelman syndrome model. J Clin Invest (2015) 125:2069–76. doi:10.1172/JCI80554
32. Sonzogni, M, Hakonen, J, Bernabé Kleijn, M, Silva-Santos, S, Judson, MC, Philpot, BD, et al. Delayed loss of UBE3A reduces the expression of Angelman syndrome-associated phenotypes. Mol Autism (2019) 10:23. doi:10.1186/s13229-019-0277-1
33. Clement, JP, Aceti, M, Creson, TK, Ozkan, ED, Shi, Y, Reish, NJ, et al. Pathogenic SYNGAP1 mutations impair cognitive development by disrupting the maturation of dendritic spine synapses. Cell (2012) 151:709–23. doi:10.1016/j.cell.2012.08.045
34. Molero, AE, Arteaga-Bracho, EE, Chen, CH, Gulinello, M, Winchester, ML, Pichamoorthy, N, et al. Selective expression of mutant huntingtin during development recapitulates characteristic features of Huntington’s disease. Proc Natl Acad Sci U S A (2016) 113:5736–41. doi:10.1073/pnas.1603871113
35. Valassina, N, Brusco, S, Salamone, A, Serra, L, Luoni, M, Giannelli, S, et al. Scn1a gene reactivation after symptom onset rescues pathological phenotypes in a mouse model of Dravet syndrome. Nat Commun (2022) 13:161. doi:10.1038/s41467-021-27837-w
36. Du, F, Nguyen, MVC, Karten, A, Felice, CA, Mandel, G, and Ballas, N. Acute and crucial requirement for MeCP2 function upon transition from early to late adult stages of brain maturation. Hum Mol Genet (2016) 25:1690–702. doi:10.1093/hmg/ddw038
37. Li, J, Levin, DS, Kim, AJ, Pappas, SS, and Dauer, WT. TorsinA restoration in a mouse model identifies a critical therapeutic window for DYT1 dystonia. J Clin Invest (2021) 131:e139606. doi:10.1172/JCI139606
38. Erro, R, Monfrini, E, and Di Fonzo, A. Chapter Ten - early-onset inherited dystonias versus late-onset idiopathic dystonias: same or different biological mechanisms? Int Rev Neurobiol (2023) 169:329–46. doi:10.1016/bs.irn.2023.05.002
39. Houlden, H, Schneider, SA, Paudel, R, Melchers, A, Schwingenschuh, P, Edwards, M, et al. THAP1 mutations (DYT6) are an additional cause of early-onset dystonia. Neurology (2010) 74:846–50. doi:10.1212/WNL.0b013e3181d5276d
40. Yellajoshyula, D, Liang, CC, Pappas, SS, Penati, S, Yang, A, Mecano, R, et al. The DYT6 dystonia protein THAP1 regulates myelination within the oligodendrocyte lineage. Dev Cel (2017) 42:52–67. doi:10.1016/j.devcel.2017.06.009
41. Goodman, CS, and Shatz, CJ. Developmental mechanisms that generate precise patterns of neuronal connectivity. Cell (1993) 72:77–98. doi:10.1016/s0092-8674(05)80030-3
42. Knudsen, EI. Sensitive periods in the development of the brain and behavior. J Cogn Neurosci (2004) 16:1412–25. doi:10.1162/0898929042304796
43. Hubel, DH, and Wiesel, TN. The period of susceptibility to the physiological effects of unilateral eye closure in kittens. J Physiol (1970) 206:419–36. doi:10.1113/jphysiol.1970.sp009022
44. Fagiolini, M, Pizzorusso, T, Berardi, N, Domenici, L, and Maffei, L. Functional postnatal development of the rat primary visual cortex and the role of visual experience: dark rearing and monocular deprivation. Vis Res (1994) 34:709–20. doi:10.1016/0042-6989(94)90210-0
45. Popescu, MV, and Polley, DB. Monaural deprivation disrupts development of binaural selectivity in auditory midbrain and cortex. Neuron (2010) 65:718–31. doi:10.1016/j.neuron.2010.02.019
46. Walton, KD, Lieberman, D, Llinás, A, Begin, M, and Llinás, RR. Identification of a critical period for motor development in neonatal rats. Neuroscience (1992) 51:763–7. doi:10.1016/0306-4522(92)90517-6
47. Inglis, FM, Zuckerman, KE, and Kalb, RG. Experience-dependent development of spinal motor neurons. Neuron (2000) 26:299–305. doi:10.1016/s0896-6273(00)81164-2
48. Warm, D, Bassetti, D, Schroer, J, Luhmann, HJ, and Sinning, A. Spontaneous activity predicts survival of developing cortical neurons. Front Cel Develop Biol (2022) 10:937761. doi:10.3389/fcell.2022.937761
49. Johnson, SL, Kuhn, S, Franz, C, Ingham, N, Furness, DN, Knipper, M, et al. Presynaptic maturation in auditory hair cells requires a critical period of sensory-independent spiking activity. Proc Natl Acad Sci U S A (2013) 110:8720–5. doi:10.1073/pnas.1219578110
50. Giachello, CNG, and Baines, RA. Inappropriate neural activity during a sensitive period in embryogenesis results in persistent seizure-like behavior. Curr Biol (2015) 25:2964–8. doi:10.1016/j.cub.2015.09.040
51. O’Donovan, MJ. The origin of spontaneous activity in developing networks of the vertebrate nervous system. Curr Opin Neurobiol (1999) 9:94–104. doi:10.1016/s0959-4388(99)80012-9
52. Hanson, MG, and Landmesser, LT. Normal patterns of spontaneous activity are required for correct motor axon guidance and the expression of specific guidance molecules. Neuron (2004) 43:687–701. doi:10.1016/j.neuron.2004.08.018
53. Warp, E, Agarwal, G, Wyart, C, Friedmann, D, Oldfield, CS, Conner, A, et al. Emergence of patterned activity in the developing zebrafish spinal cord. Curr Biol (2012) 22:93–102. doi:10.1016/j.cub.2011.12.002
54. Myers, CP, Lewcock, JW, Hanson, MG, Gosgnach, S, Aimone, JB, Gage, FH, et al. Cholinergic input is required during embryonic development to mediate proper assembly of spinal locomotor circuits. Neuron (2005) 46:37–49. doi:10.1016/j.neuron.2005.02.022
55. Zeng, X, Komanome, Y, Kawasaki, T, Inada, K, Jonaitis, J, Pulver, SR, et al. An electrically coupled pioneer circuit enables motor development via proprioceptive feedback in Drosophila embryos. Curr Biol (2021) 31:5327–40.e5. doi:10.1016/j.cub.2021.10.005
56. Carreira-Rosario, A, York, RA, Choi, M, Doe, CQ, and Clandinin, TR. Mechanosensory input during circuit formation shapes Drosophila motor behavior through patterned spontaneous network activity. Curr Biol (2021) 31:5341–9.e4. doi:10.1016/j.cub.2021.08.022
57. Hunter, I, Coulson, B, Pettini, T, Davies, JJ, Parkin, J, Landgraf, M, et al. Balance of activity during a critical period tunes a developing network. Preprint (2023). Available from: https://doi.org/10.1101/2023.08.04.551972.
58. Lybrand, ZR, Goswami, S, Zhu, J, Jarzabek, V, Merlock, N, Aktar, M, et al. A critical period of neuronal activity results in aberrant neurogenesis rewiring hippocampal circuitry in a mouse model of epilepsy. Nat Commun (2021) 12:1423. doi:10.1038/s41467-021-21649-8
59. Larkby, C, and Day, N. The effects of prenatal alcohol exposure. Alcohol Health Res World (1997) 21:192–8.
60. Hensch, TK, and Quinlan, EM. Critical periods in amblyopia. Vis Neurosci (2018) 35:E014. doi:10.1017/S0952523817000219
61. Niparko, JK, Tobey, EA, Thal, DJ, Eisenberg, LS, Wang, NY, Quittner, AL, et al. Spoken Language development in children following cochlear implantation. JAMA (2010) 303:1498–506. doi:10.1001/jama.2010.451
62. LeBlanc, JJ, and Fagiolini, M. Autism: a ‘critical period’ disorder? Neural Plast (2011) 2011:921680. doi:10.1155/2011/921680
63. Selemon, LD, and Zecevic, N. Schizophrenia: a tale of two critical periods for prefrontal cortical development. Transl Psychiatry (2015) 5:e623. doi:10.1038/tp.2015.115
Keywords: dystonia, critical periods, neurodevelopment, drosophila, BK channels
Citation: Lowe SA (2024) Critical developmental periods of vulnerability in dystonia. Dystonia 3:12125. doi: 10.3389/dyst.2024.12125
Received: 26 September 2023; Accepted: 04 March 2024;
Published: 13 March 2024.
Edited by:
G. W. Gant Luxton, University of California, Davis, United StatesCopyright © 2024 Lowe. This is an open-access article distributed under the terms of the Creative Commons Attribution License (CC BY). The use, distribution or reproduction in other forums is permitted, provided the original author(s) and the copyright owner(s) are credited and that the original publication in this journal is cited, in accordance with accepted academic practice. No use, distribution or reproduction is permitted which does not comply with these terms.
*Correspondence: Simon A. Lowe, s.lowe@ucl.ac.uk